Infrared spectroscopy (IR) is a versatile analytical technique used to study the vibrational and rotational transitions of molecules in the infrared region of the electromagnetic spectrum. It involves the absorption, emission, or reflection of infrared radiation by molecules, providing valuable information about their chemical structure, functional groups, and bonding characteristics. By measuring the frequencies at which molecules absorb infrared radiation, IR spectroscopy enables the identification of compounds and the qualitative and quantitative analysis of their chemical composition.
Theory of Infrared Spectroscopy
Infrared spectroscopy is a widely used analytical technique based on the principle that molecules absorb infrared radiation in the mid-infrared region of the electromagnetic spectrum, typically spanning wavelengths from 2.5 to 25 micrometers (or wavenumbers ranging from 4000 to 400 cm⁻¹). This absorption occurs due to the excitation of molecular vibrational and rotational modes, which are characteristic of the chemical bonds and functional groups present in a molecule. The fundamental theory of Infrared spectroscopy revolves around the interaction of infrared radiation with molecular bonds, providing crucial information about molecular structure, bond types, and chemical environments.
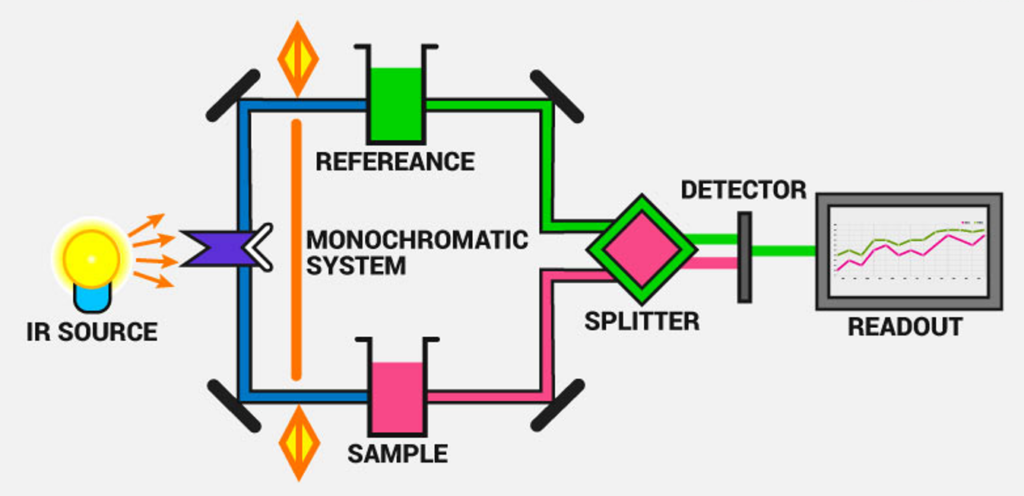
Vibrational Modes in Infrared spectroscopy
Molecules are composed of atoms held together by chemical bonds, which act like elastic springs and can undergo periodic vibrations. These vibrations can be classified into two main types:
- Stretching Vibrations: These involve changes in the bond length between two atoms. Stretching can be further categorized into:
Symmetric Stretching: Both atoms move in and out along the bond axis simultaneously.
Asymmetric Stretching: One atom moves in while the other moves out, leading to an unsymmetrical change in bond length.
- Bending Vibrations: These involve changes in the bond angle and can be classified into:
Scissoring: Two atoms move toward or away from each other in the same plane.
Rocking: Atoms move in the same direction while maintaining their bond length.
Wagging: Atoms move in opposite directions perpendicular to the molecular plane.
Twisting: Atoms rotate around the bond axis in opposite directions.
Each vibrational mode has a unique frequency or wavenumber, which is expressed in reciprocal centimeters (cm⁻¹). The absorption of IR radiation at specific frequencies corresponds to these vibrational modes and provides a fingerprint of the molecular structure.
Rotational Modes in Infrared spectroscopy
In addition to vibrational motions, molecules can also undergo rotational transitions, wherein the entire molecule rotates around its center of mass. Rotational transitions occur at much lower energies compared to vibrational transitions and are generally observed in the far-infrared region of the spectrum. However, rotational spectra are more relevant to gas-phase molecules and are not typically considered in conventional Infrared spectroscopy of solids and liquids.
Selection Rules in Infrared spectroscopy
For a molecular vibration to be IR-active, it must obey the selection rules of infrared spectroscopy. The primary rule states that a vibrational transition is allowed only if there is a change in the dipole moment of the molecule during the vibration. This means:
- Molecules with permanent dipole moments (e.g., water, carbon dioxide, and alcohols) absorb IR radiation effectively and exhibit strong IR spectra.
- Symmetric molecules (e.g., homonuclear diatomic molecules like N₂ and O₂) do not exhibit IR absorption because their vibrations do not induce a change in dipole moment, making them IR-inactive.
Additionally, symmetric stretching vibrations often do not appear in IR spectra because they do not result in a net change in dipole moment.
Absorption of Infrared Radiation and Molecular Excitation
When a molecule absorbs infrared radiation of a specific energy, it transitions from its ground vibrational state to an excited vibrational state. The absorbed energy must match the energy difference between these states, which is determined by the vibrational frequency of the molecular bond. The relationship between energy (E) and frequency (ν) is given by Planck’s equation:
where is Planck’s constant (6.626 × 10⁻³⁴ Js) and is the vibrational frequency. The absorbed IR radiation thus provides a spectrum that represents the unique vibrational fingerprints of a molecule, which can be used for qualitative and quantitative analysis.
Beer-Lambert Law and Quantitative Analysis
The intensity of IR radiation absorbed by a sample follows the Beer-Lambert law, which describes the relationship between absorbance (A), concentration (c), and path length (l) of the sample. It is mathematically expressed as:
where:
- = Absorbance (unitless)
- = Molar absorptivity (L mol⁻¹ cm⁻¹), a measure of how strongly a substance absorbs IR radiation at a specific wavelength
- = Concentration of the absorbing species (mol/L)
- = Path length of the sample (cm)
The Beer-Lambert law allows Infrared spectroscopy to be used not only for identifying molecular structures but also for determining the concentration of specific functional groups within a sample.
Types of Infrared spectroscopy
Infrared spectroscopy encompasses various techniques tailored to specific experimental requirements and sample types. These techniques differ in measurement principles, sample handling, and spectral acquisition. Below are detailed notes on the types of IR spectroscopy:
1. Transmission Infrared Spectroscopy
Principle: Transmission Infrared Spectroscopy is a widely used analytical technique that measures the amount of infrared radiation passing through a sample. This method is based on the fundamental principle that different molecular bonds absorb infrared radiation at characteristic wavelengths, leading to molecular vibrations. The extent of absorption at specific wavelengths provides valuable information about the functional groups present within the sample.
The technique follows the Beer-Lambert Law, which establishes a direct relationship between the absorbance of IR radiation, the concentration of the absorbing species, and the path length through which the IR beam travels. This principle allows for qualitative and quantitative analysis of a wide range of materials, including organic and inorganic compounds.
Instrumentation of Transmission Infrared Spectroscopy
A standard transmission IR spectrometer consists of several key components that work together to generate, direct, and analyze infrared radiation. These components include:
- Infrared Radiation Source: Typically, a heated ceramic, Nernst glower, or Globar (silicon carbide) serves as the IR source, emitting a broad range of infrared wavelengths.
- Sample Compartment: The sample is placed in a specialized holder or cell that is transparent to IR radiation. Materials such as sodium chloride (NaCl) or potassium bromide (KBr) are commonly used for this purpose, as they do not absorb IR radiation within the measured range.
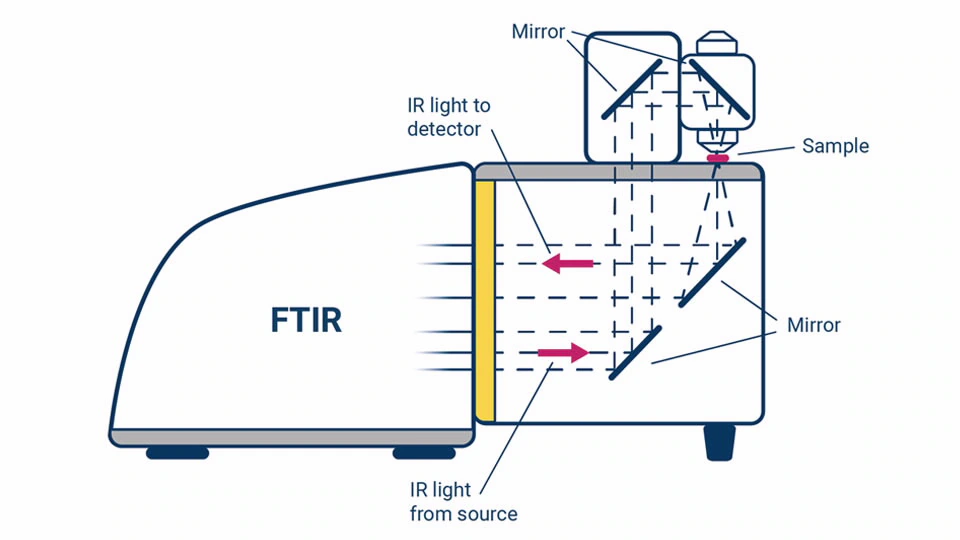
- Monochromator: This component functions to selectively filter and isolate specific wavelengths of IR radiation, ensuring accurate spectral analysis by preventing interference from undesired wavelengths.
- Detector: Common detectors include pyroelectric detectors, thermocouples, or mercury cadmium telluride (MCT) detectors, which convert the absorbed IR radiation into an electrical signal for further analysis.
- Data Processing System: The final component of the system is a computer-based interface that collects the detected signals, processes the data, and generates an IR absorption spectrum. This spectrum represents the transmittance (or absorbance) of the sample as a function of wavelength, aiding in compound identification.
Advantages of Transmission Infrared spectroscopy:
- High Sensitivity and Accuracy: Transmission Infrared spectroscopy is a well-established and highly sensitive analytical technique capable of detecting minute quantities of a substance.
- Versatile Application Across Different Phases of Matter: This method is suitable for the analysis of a wide variety of sample types, including liquids, gases, and thin solid films.
- Reliable and Reproducible Results: Due to its robust methodology and extensive historical use, transmission IR spectroscopy provides highly reproducible and reliable spectral data for material characterization.
Limitations of Transmission Infrared spectroscopy:
- Sample Transparency Requirement: The sample must be sufficiently transparent to IR radiation. Opaque or highly scattering materials may not be suitable for direct transmission measurements, necessitating alternative IR techniques such as Attenuated Total Reflectance (ATR).
- Physical Constraints on Sample Preparation: The sample must be prepared in an appropriate form, such as a thin film or a dissolved solution, to allow effective transmission of IR radiation. This requirement may limit the applicability of the technique to certain types of solid samples with low solubility or high thickness.
- Influence of Atmospheric Interference: Moisture and carbon dioxide present in the surrounding atmosphere can sometimes interfere with IR measurements, affecting spectral accuracy. Proper purging of the system with dry nitrogen or argon gas is often necessary to minimize such interferences.
2. Attenuated Total Reflection (ATR) Infrared Spectroscopy
Principle: Attenuated Total Reflection (ATR) Infrared (IR) Spectroscopy is an advanced variation of traditional IR spectroscopy that enables the analysis of a wide range of samples with minimal preparation. This technique measures the absorbance of infrared radiation that is reflected at the interface between a sample and an internal reflection element (IRE).
The key principle behind ATR spectroscopy is total internal reflection, which occurs when an IR beam passes from a dense medium (the IRE crystal) into a less dense medium (the sample) at an angle exceeding the critical angle. This results in the formation of an evanescent wave, a type of electromagnetic field that extends a short distance beyond the surface of the crystal into the sample. The evanescent wave interacts with the sample, allowing selective absorption of IR radiation at characteristic frequencies corresponding to the sample’s molecular vibrations. The absorbed IR radiation is then analyzed to produce an IR spectrum, providing molecular-level information about the sample composition.
This method significantly enhances sensitivity and allows for non-destructive analysis of various sample types, including solids, liquids, and powders, without extensive sample preparation.
Instrumentation:
The instrumentation used in ATR-IR spectroscopy is similar to that of conventional transmission IR spectroscopy, with the primary difference being the inclusion of an ATR accessory. The major components of an ATR-IR spectrometer include:
- Infrared Radiation Source: A heated element (such as a Globar or Nernst glower) generates a broad spectrum of infrared radiation.
- ATR Accessory: The ATR setup is the defining feature of this technique and consists of:
- Internal Reflection Element (IRE) or ATR Crystal: A high-refractive-index material such as diamond, germanium (Ge), zinc selenide (ZnSe), or silicon (Si) is used as the ATR crystal. The IR beam passes through this crystal, undergoing multiple internal reflections.
- Sample Contact Surface: The sample is placed in direct contact with the ATR crystal, ensuring efficient interaction between the evanescent wave and the sample molecules.
- Monochromator or Interferometer: Filters and selects specific IR wavelengths for analysis, ensuring accurate spectral data collection.
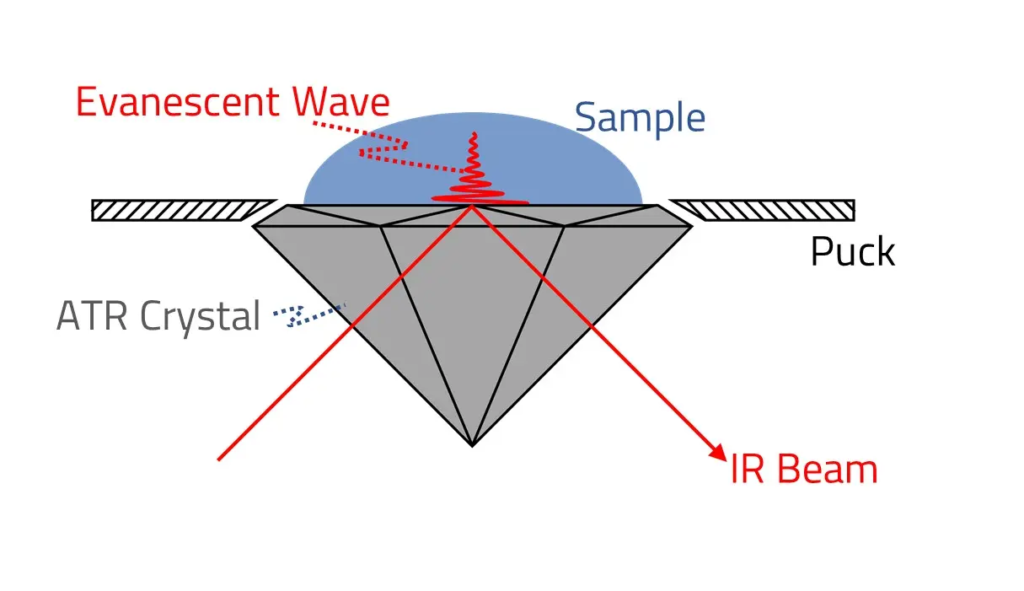
- Detector: Commonly used detectors include pyroelectric, thermoelectric, or mercury cadmium telluride (MCT) detectors, which convert IR absorption into an electrical signal for processing.
- Data Processing System: The final spectral data is recorded and processed using specialized software to generate an IR absorption spectrum, revealing detailed molecular fingerprinting of the sample.
Advantages of ATR-IR Spectroscopy:
- Minimal Sample Preparation: Unlike traditional transmission IR spectroscopy, ATR does not require the preparation of thin films, pellets, or solutions. Solid and liquid samples can be analyzed directly, saving time and effort.
- Versatile Sample Compatibility: ATR is suitable for analyzing a broad range of samples, including solids (such as polymers, tablets, and coatings), liquids, gels, and even powders, making it highly versatile for various analytical applications.
- Non-Destructive and Reusable Sample Handling: Since ATR-IR analysis does not significantly alter the sample, it can be retrieved for further testing or additional analysis if required.
- Enhanced Spectral Reproducibility and Accuracy: The direct contact of the sample with the ATR crystal ensures consistent spectral data, improving reproducibility and reliability.
Limitations of ATR-IR Spectroscopy:
- Limited Penetration Depth into the Sample: The evanescent wave typically penetrates only a few micrometers (usually 0.5 to 5 µm) into the sample, meaning that ATR primarily provides surface-layer information. This limitation may hinder bulk composition analysis, particularly for heterogeneous materials.
- Potential Interactions with the ATR Crystal: Some samples may chemically react with or adhere to the ATR crystal surface, leading to potential spectral distortions. Proper selection of the ATR crystal material is necessary to minimize these interactions.
- Dependence on Sample Contact: Effective ATR analysis requires strong and uniform contact between the sample and the ATR crystal. Soft, irregular, or non-uniform samples may produce inconsistent spectral results due to poor contact.
3. Diffuse Reflectance Infrared (IR) Spectroscopy (DRIFTS)
Principle: Diffuse Reflectance Infrared Spectroscopy (DRIFTS) is a specialized IR technique used to analyze solid samples, particularly powders and materials with rough surfaces. Instead of measuring transmitted IR radiation (as in transmission IR spectroscopy) or internal reflection (as in ATR-IR spectroscopy), DRIFTS measures the IR radiation reflected from the surface of a sample.
When infrared light interacts with a powdered or rough-surfaced sample, some of the incident radiation is absorbed, while the remaining portion is scattered in multiple directions. This scattered radiation consists of two main components:
- Specular Reflection: A mirror-like reflection that follows the laws of geometric optics, contributing minimal analytical value.
- Diffuse Reflection: A scattered, multi-directional reflection resulting from multiple interactions with the sample particles. This diffuse reflection carries valuable spectral information and forms the basis of DRIFTS analysis.
By capturing and analyzing the diffusely reflected IR radiation, DRIFTS enables the identification of functional groups and molecular structures within a sample, making it an effective technique for studying complex solid materials.
Instrumentation:
The instrumentation for diffuse reflectance Infrared spectroscopy is similar to conventional IR spectroscopy, with the key addition of a diffuse reflectance accessory that enhances the detection of scattered IR radiation. The main components of a DRIFTS system include:
- Infrared Radiation Source: A standard IR source, such as a Globar (silicon carbide) or Nernst glower, emits infrared radiation.
- Diffuse Reflectance Accessory: This specialized attachment directs the IR beam toward the sample and collects the scattered radiation. The sample is typically finely ground and mixed with a non-absorbing matrix material (such as potassium bromide (KBr) or barium sulfate (BaSO₄)) to optimize reflection and minimize strong absorption bands.
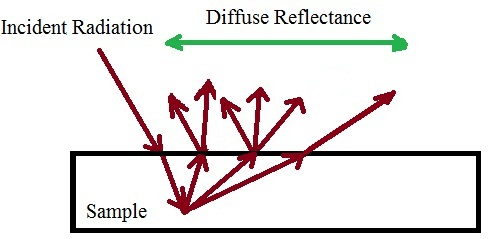
- Sample Compartment: The powdered sample is either packed onto a rough surface or mixed with a diluting medium to ensure consistent reflectance. In some cases, a special sample cup or holder is used to contain the material while allowing optimal IR interaction.
- Monochromator or Interferometer: A spectral filtering system isolates specific IR wavelengths for analysis, preventing interference from unwanted signals.
- Detector: Commonly used MCT (Mercury Cadmium Telluride) or deuterated triglycine sulfate (DTGS) detectors convert the reflected IR signal into an electrical response for data processing.
- Data Processing System: The collected spectral data is processed and analyzed using computer software to generate an absorption spectrum, allowing for material identification and characterization.
Advantages of Diffuse Reflectance IR Spectroscopy (DRIFTS):
- Ideal for Solid Sample Analysis: DRIFTS is particularly well-suited for studying powders, heterogeneous materials, and rough surfaces, making it valuable for applications in pharmaceuticals, catalysis, mineralogy, and polymer science.
- Minimal Sample Preparation Required: Unlike transmission IR spectroscopy, DRIFTS does not require pellet formation or thin film preparation. Instead, powdered samples can be analyzed directly, saving time and effort.
- Non-Destructive Technique: Since no chemical alteration of the sample occurs, the technique allows for further testing or reanalysis of the same sample.
- Compatible with a Wide Range of Materials: DRIFTS can analyze both organic and inorganic compounds, including minerals, catalysts, pharmaceuticals, and polymeric materials.
Limitations of Diffuse Reflectance Infrared spectroscopy (DRIFTS):
- Lower Sensitivity Compared to Transmission and ATR-IR Spectroscopy: The intensity of diffusely reflected IR radiation is often weaker than transmitted IR signals, leading to lower spectral resolution and sensitivity.
- Variability Due to Surface Properties and Sample Packing: Surface roughness, particle size distribution, and sample packing density can significantly affect spectral reproducibility. Variations in these factors may introduce inconsistencies in the obtained spectra.
- Requirement for a Non-Absorbing Dilution Medium: Strongly absorbing samples may require mixing with a non-absorbing powder (e.g., KBr or BaSO₄) to enhance reflection and prevent total absorption, which can complicate sample preparation.
4. Fourier Transform Infrared (FT-IR) Microscopy
Principle: FT-IR Microscopy is an advanced technique that combines the capabilities of an optical microscope with a Fourier Transform Infrared (FT-IR) spectrometer, allowing for highly localized, spatially resolved IR analysis of microscopic samples. This method enables researchers to obtain chemical and structural information from extremely small sample areas, making it useful for investigating heterogeneous materials, contaminant identification, and forensic analysis.
FT-IR microscopy works by focusing infrared radiation onto a specific microscopic region of interest and measuring the IR absorbance or reflectance at that precise location. The resulting spectra provide molecular fingerprints of the sample, revealing details about its composition, functional groups, and structural properties.
Instrumentation:
The key components of an FT-IR microscopy system include:
- FT-IR Spectrometer: A Fourier Transform Infrared Spectrometer serves as the primary detection unit, collecting IR spectra from the sample.
- Optical Microscope Coupled with FT-IR System: A high-precision optical microscope is integrated with the FT-IR spectrometer, enabling microscopic visualization and alignment of the sample.
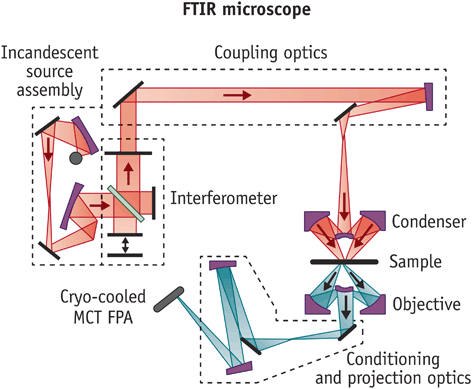
- IR-Transparent Sample Stage or Slide: The sample is typically mounted on a CaF₂ (calcium fluoride), ZnSe (zinc selenide), or BaF₂ (barium fluoride) window, which is transparent to infrared radiation to minimize background interference.
- Condensed and Focused IR Beam: A Cassegrain objective or an ATR objective lens is used to focus the IR beam onto a very small region of the sample.
- High-Sensitivity IR Detector: Detectors such as Mercury Cadmium Telluride (MCT) are used due to their high sensitivity and ability to capture detailed spectral data.
Advantages of FT-IR Microscopy:
- Ideal for Small or Heterogeneous Samples: Unlike conventional IR techniques that require bulk material, FT-IR microscopy enables the analysis of microscopic sample areas, making it suitable for forensics, biomedical research, and pharmaceutical applications.
- Provides Spatially Resolved Chemical Information: This technique allows for mapping chemical composition across a sample, making it highly valuable in material characterization, failure analysis, and quality control.
- Non-Destructive Analysis: The method does not require extensive sample preparation, preserving the integrity of the sample for further studies.
Limitations of FT-IR Microscopy:
- Lower Sensitivity Compared to Bulk IR Spectroscopy: Because only a small amount of sample is analyzed at a time, the signal strength is weaker than traditional IR techniques, requiring highly sensitive detectors.
- Sample Preparation and Handling Can Be Challenging: Very thin sections or microtomed samples may be needed to ensure accurate spectral acquisition, which can be difficult for certain materials.
5. Reflectance Infrared Spectroscopy
Principle: Reflectance Infrared Spectroscopy is a technique that analyzes the infrared radiation reflected from a sample surface rather than transmitted through it. This technique is particularly useful for studying solid surfaces, thin films, and coatings that cannot be easily prepared for transmission IR spectroscopy.
Reflectance Infrared spectroscopy works on two main reflection principles:
- Specular Reflection: Occurs when IR radiation is reflected in a mirror-like manner from a smooth surface. This method is particularly useful for analyzing thin films and coatings.
- Diffuse Reflection (DRIFTS): Occurs when IR radiation is scattered in multiple directions from a rough or powdered surface. This technique is commonly used for solid-state materials and powders.
Depending on the nature of the sample, either specular or diffuse reflectance mode is used to obtain spectral information about surface composition, chemical functionality, and molecular interactions.
Instrumentation:
A typical Reflectance Infrared spectroscopy setup includes:
- Infrared Radiation Source: A standard Globar (silicon carbide) or Nernst glower provides a broad IR spectrum for analysis.
- Reflectance Accessory: The setup includes a specialized reflectance module that directs IR light onto the sample surface and collects the reflected radiation at an optimal angle.
- Sample Stage: The sample is positioned at a specific angle to maximize reflectance efficiency. Highly reflective substrates, such as gold or aluminum-coated slides, may be used to enhance signal strength.
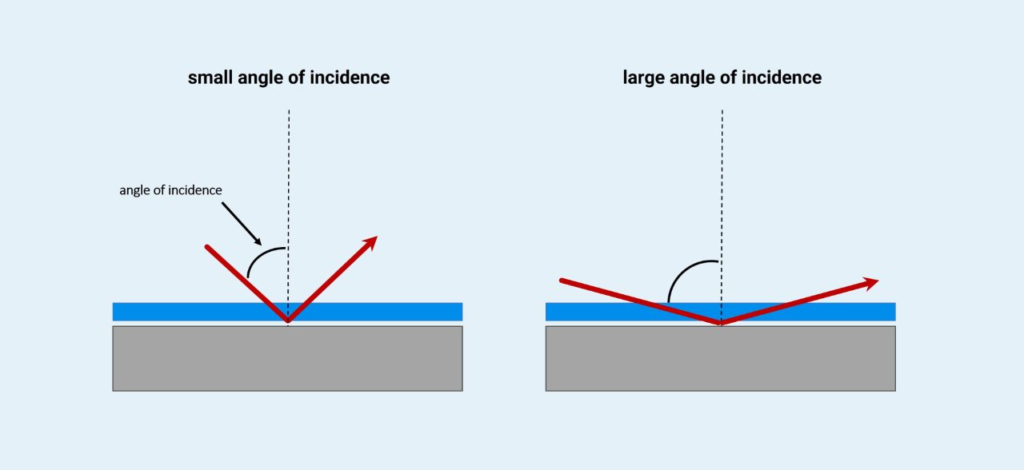
- Monochromator or Interferometer: Used to isolate specific IR wavelengths for accurate spectral acquisition.
- IR Detector: High-sensitivity detectors such as MCT (Mercury Cadmium Telluride) or DTGS (Deuterated Triglycine Sulfate) are used to capture the reflected signal.
Advantages of Reflectance Infrared spectroscopy:
- Suitable for Thin Films, Coatings, and Surface Analysis: This technique is highly effective for analyzing surface properties, making it valuable in industries such as semiconductors, coatings, and materials science.
- Minimal Sample Preparation: Unlike transmission IR, which requires thin pellets or solutions, reflectance IR can be performed on intact surfaces without altering the sample.
- Can Be Used for Non-Destructive Testing (NDT): Reflectance IR is ideal for analyzing historical artifacts, artwork, or biological samples where preserving the original material is crucial.
Limitations of Reflectance Infrared spectroscopy:
- Less Sensitive Than Transmission and ATR Techniques: Because only a fraction of the incident IR radiation is reflected, the signal strength is lower than techniques that rely on direct absorption.
- Surface Properties and Roughness Can Affect Spectra: Highly polished surfaces produce strong specular reflection, while rough or uneven surfaces may cause unwanted scattering and distort spectral data.
Each type of Infrared spectroscopy offers unique advantages and is suited to different sample types and experimental requirements. Understanding the principles and applications of these techniques is essential for selecting the most appropriate method for a given analytical task. By harnessing the power of IR radiation, scientists can gain valuable insights into the chemical composition, structure, and properties of diverse materials.